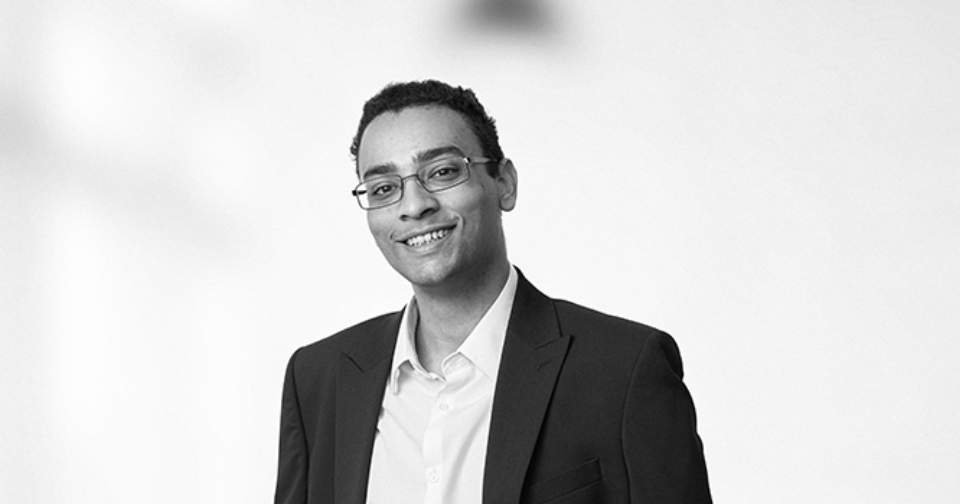
Space-borne sensors serve a variety of purposes that are all but essential to our daily lives, from GPS navigation to weather prediction. They also play a key role in the ongoing battle against climate change. For example, space-borne sensors can determine atmospheric pollution levels, monitor global temperatures on the land and in the sea, and track climate-related processes such as deforestation. Every year we launch more and more of these sensors into orbit in the hope of returning ever more accurate results, but we are now reaching the limits of what classical instruments can offer us. As a result, people are starting to look at quantum physics as a way to keep development moving forward.
One, slightly surprising, way that scientists can track the effects of climate change is by using space-borne sensors to measure the Earth’s gravitational field. This is because variations in the strength of the gravitational field across the Earth’s surface can be used to determine points of more or less concentrated mass, which may correspond to ice sheets, activity in the mantle or even mineral deposits in the Earth’s crust. Further, by tracking changes in the gravitational field over time, scientists can accurately record and analyse the movement of large quantities of mass. An example being the redistribution of water across the globe caused by melting polar ice caps.
In recent years, there have been two main experiments carried out to study Earth’s gravitational field from space. The first of these was known as the Gravity Recovery and Climate Experiment (GRACE), which used small deviations in the distance between two identical orbiting satellites to detect gravitational anomalies. I.e., points where the gravitational field differs from the average. By tracking the movement of these anomalies over the 2002-2017 lifespan of the mission, scientists were able to detect changes in the distribution of the water across the planet, measure ocean currents, and record mass loss within ice sheets in Greenland and Antarctica. In fact, this data was so useful that the GRACE Follow On (GRACE FO) mission was commissioned to run between 2018-2023, which uses two new and improved satellites to continue where the GRACE satellites left off.
The second experiment was the Gravity Field and Steady-State Ocean Circulation Explorer (GOCE), which ran between 2009-2013. Rather than tracking gravitational anomalies over time, the GOCE mission instead aimed to map Earth’s gravitational field by using three orthogonal sets of accelerometers to measure spatial variations in the field’s strength. The result was the most accurate and highly resolved geoid (i.e., a model showing what the Earth would look like if its entire surface was covered in one big ocean) ever created, which has provided us with a better understanding of the Earth’s interior and better estimates of the thickness of polar ice sheets.
But whilst both of these missions have been incredibly important in pushing forward our understanding of the Earth and the effect we are having on it, there are limits on what the classical instruments used in GRACE and GOCE can provide. For example, classical accelerometers have large long-term drifts (caused in part by mechanical wear and tear), which will decrease the accuracy of the results over time and make it more difficult to model temporal fluctuations. Moreover, the sensitivity and resolution of classical instruments is limited by noise considerations in the measurements, which will make it difficult to achieve more accurate results (see Quantum technologies in space).
One way of improving upon the results of GRACE and GOCE is to use quantum, rather than classical, instruments like cold atom interferometers. Now in a boring, non-quantum, interferometer, you take a beam of light, split the beam (using mirrors) along two different paths, recombine the two beams (using more mirrors) at a later point, then make a measurement to determine a ‘phase difference’ between the light that followed the first and second paths. Don’t worry, knowing what is meant by phase difference isn’t particularly important here, just know that it is proportional to the quantity that the user is attempting to measure (for example, gravity).
A cold atom interferometer works on this same principle, but with a couple of important differences. The first difference is that a cold atom interferometer flips the respective roles of matter and light; using one or more atoms in place of the beam of light, and laser beams in place of mirrors. More specifically, a cold atom interferometer manipulates the one or more atoms using three precisely timed lasers: one to split, one to recombine, and one to mix (this mixing concept will be made clearer below). The second difference is the quantum mechanical nature of the cold atom interferometer, which lies at the heart of why I can say ONE or more atoms being split, recombined and mixed.
You see, quantum objects can exist in multiple states simultaneously just so long as they aren’t measured. Think of Schrodinger stuffing his cat in a box with some poison that could be released at any moment. It’s not simply that we don’t know whether this quantum cat is alive or dead (because the box is closed for instance), but that it is literally alive and dead at the same time. In other words, it exists in a quantum mechanical superposition of the alive and dead states, and it is only when we open the box (i.e., measure the system) that the cat collapses to one or the other of the possible options.
Taking things back to the cold atom interferometer working with a single atom, the first laser pulse is precisely calibrated to have a 50% chance of transitioning the atom into an exited state. As a result, the atom is moved into a quantum mechanical superposition in which it exists in both an exited and ground state simultaneously, with equal probability of collapsing to either state if we were to measure the system at this point. It’s worth reiterating though that just because we can’t measure these two component states without collapsing things, they both really do exist simultaneously. Moreover, these two (really real) component states are now moving along different physical paths in space, and so gravity is acting on them differently just as it did with the two split beams of light.
The second pulse is configured to bring the split states of the atom back to the same point in space, having a 100% chance to transition the atom from whatever state it is in into the other state. In other words, the exited component state transitions to the ground state, and the ground component state transitions to the exited state, giving what was the ground component state the chance to catch up to what was the exited component state. In effect then, the atom was split into two component states at the start, with each following a different path, and now, thanks to the second laser pulse, these two component states have been put onto a collision course back together again.
This is where the third laser pulse comes into play, its purpose being to ‘interact’ the two possible component states of the atom wavefunction at the exact moment that the paths of the two components collide. Each of these components has an equal weight in this interaction process as there was an equal chance of the atom being measured in either component state at any point throughout. But, thanks to the effect of gravity, each of the two possible components has a different phase, and the third laser interaction step ensures that this phase difference is reflected in the final atomic wavefunction (which will be a mix of the ground and exited states). Measuring the final state of the atom after this third laser pulse will thus provide data on the gravitational force acting on the interferometer system (i.e., provide a gravitational measurement).
But using only one atom, all you will have at the end is a single atom measured to be in either the exited or ground state. This doesn’t provide much information about the phase difference that developed between the two component paths during the process, and so also tells you very little about gravitational strength. So instead, whole clouds of atoms are sent through the interferometer at a time, with each constituent atom being at a very low temperature to prevent the clouds from dispersing too much halfway through the process (hence the ‘cold’ atom interferometer name). In this way, gravitational strength can be measured, and the result made more accurate with more atoms.
‘Okay, that’s all well and good, but what’s the advantage of going to all the extra trouble of using these one or more atoms over a light beam?’ you might ask. Well, in an interferometer, the longer that the beam of light/group of atoms spend split apart along the two different paths, the bigger the phase difference between them. This is important because the sensitivity of the instrument is proportional to the square of the time that the beams spend separated (i.e., doubling the split time quadruples the sensitivity), and so keeping the atoms apart for longer means more accurate measurements. But light travels at approximately 300,000,000 m/s, meaning that highly sensitive classical interferometers need to be incredibly big to keep two beams separated for longer periods of time (see, for example, LIGO in the US, which was used to detect the first gravitational waves). Therefore, even though a classical interferometer can be very sensitive, getting it into space in the first place would be extremely difficult.
In contrast, atom interferometers use atoms rather than light, which move around a lot more slowly. Better still, the atoms used in cold atom interferometers are cooled down to very low temperatures, reducing their speed even more. This means that the atoms can be split apart for long periods of time even in small instruments, making cold atom interferometer’s very attractive for gravitational measurements.
The value of cold atom interferometers to space-borne sensing has been recognised since at least 2010 when this technology was mentioned in the European Space Agency’s ‘Roadmap for Fundamental Physics in Space’, and since this time there has been a small but consistent number of patent applications relating to cold atom interferometer technology that might one day be used in space being filed annually (see the EPOs recent ‘Quantum technologies and space’ report). These filings reflect the sustained interest in the technology, as well as the ongoing efforts of science and industry to overcome the remaining challenges preventing cold atom interferometers from being used in space missions.
One recent example of a project moving things forward is the Cold Atom Space Payload (CASPA) mission, which aimed to reduce the size and weight of the device that generates the cold atoms for use in the interferometer. The device itself works in two stages. First, it uses a magnetic field generated by a series of electromagnets to trap the atoms within the device. Then, once the atoms are trapped, the device cools the atoms down using a set of six orthogonal counter propagating lasers (basically, the lasers are pointing inwards towards the six faces of an imaginary cube), where the frequency of the lasers is precisely controlled so that photons from a particular laser can only be absorbed by atoms moving toward that laser. Once a photon has been absorbed, the exited atom will then (most likely) reemit the photon in a different direction to that followed by the original photon, meaning that the atom will end up travelling toward the laser more slowly than it was originally. By cycling through this mechanism, known as Doppler cooling, many times, the speed of the atoms (and by extension their ‘temperature’) is reduced to near zero.
Now, it might be expected that a relatively complex device such as this would be quite big, and so offset some of the size advantage that the cold atom interferometer gains from using cold atoms in the first place. However, the CASPA project has been able to reduce this entire system into a 4kg payload that fits into a 20 x 20 x 10 cm space (see Gravity sensing: cold atom trap onboard a 6U CubeSat), meaning that this essential component of the cold atom interferometer can be more easily (and cheaply) transported into space.
Of course, this result is not the final piece of the puzzle for space-based cold atom interferometers, with those involved in the project themselves acknowledging that this is only an intermediate step. But what it does show is that it is more a question of when, not if, we see quantum sensors such as this being used to make gravitational measurements in space.
Jack is a patent attorney working in the Bristol office as part of the engineering and ICT team. Jack graduated from the University of Warwick with a first class Integrated Masters degree in Physics (MPhys). His final year project focused on studying the epitaxial growth and characterisation of silicon-based semiconductor materials fabricated using Chemical Vapour Deposition. During his time at Warwick, he also completed a summer research project in which he developed Python simulations of the thermodynamic properties of ideal spin-lattice materials for use in the ongoing materials research at the Universities of Warwick and Oxford.
Email: jack.davies@mewburn.com
Our IP specialists work at all stage of the IP life cycle and provide strategic advice about patent, trade mark and registered designs, as well as any IP-related disputes and legal and commercial requirements.
Our peopleWe have an easily-accessible office in central London, as well as a number of regional offices throughout the UK and an office in Munich, Germany. We’d love to hear from you, so please get in touch.
Get in touch