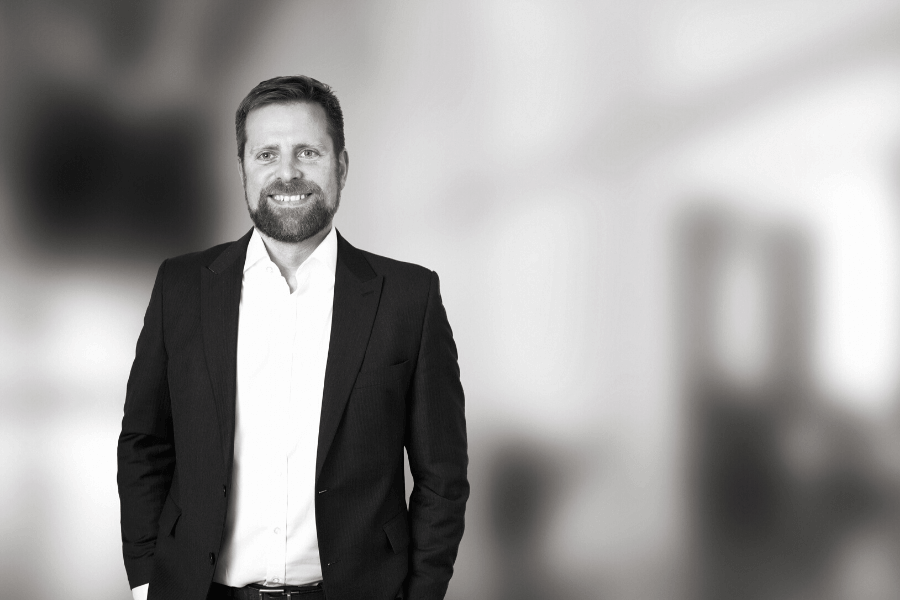
Innovation must produce sustainable solutions in every area of technology to help the world avert the most devastating extremes of the climate crisis that many are experiencing right now.
As a result of the ongoing shift in how we generate and use energy, the demand for effective electricity storage is rocketing. As detailed in our Green IP report, batteries have a key role to play as an enabling technology for the reduction of greenhouse gas emissions.
The importance of battery technology can be exemplified by observing patenting activity; a joint study between the IEA and the EPO found that, in 2018, batteries accounted for 88% of all international patenting activity in the area of electricity storage, an area that itself has seen an increase in international patent filings of 704% from 2000 to 2018.
The big name in the battery world is “Li-ion”. The (commercial) Li-ion battery celebrates its 30th birthday this year and won its inventors a Nobel prize in 2019. A familiar and accomplished technology, yes; a perfect protagonist capable of leading us into the sustainable future, possibly not.
As time goes on, standards on safety, sustainability and performance are rising and this is opening the door to new technology and innovation in all facets of the modern battery.
Whilst the Li-ion battery has come a long way since 1991, some persistent flaws include temperature and moisture sensitivity, potential flammability, and a rather unsatisfactory lifespan (as anyone with a smart phone that is a few years old can attest). These weaknesses are in part due to the nature of the electrolyte.
An electrolyte is an ion transport medium that connects the anode to the cathode – its essential feature is a high ionic conductivity and a low electronic conductivity – allowing ions (usually Li+) to pass through it when charging and discharging, but ensuring that electrons move between electrodes via our electronic devices. Standard electrolytes are generally either a non-aqueous lithium salt solution (Lithium hexafluorophosphate (LiPF6) salt dissolved in organic carbonates, for example) or a polymer gel (best thought of as a lithium salt solution contained within a polymer matrix).
An area of great interest is the use of Solid-State Electrolytes (SSEs) as replacements for the liquid (or polymer gel) electrolytes in most of today’s Li-ion batteries; the IEA report notes that the percentage of all Li-ion patenting activity directed towards solid-state battery technology (largely SSEs) went from 3% to 8% from 2010 – 2020, growing 25% year-on-year. In fact, in November, 2021, the European Commission published a roadmap on cell design and manufacturing, ultimately setting the goal for ‘generation 4’ cells from 2025 which are to be entirely solid-state.
Solid state electrolytes are capable of conducting ions through their bulk. One of the early examples of ionic conductivity in the solid state is silver iodide (AgI), a ‘textbook’ example taught in undergraduate courses the world over. Heat AgI to 146 C and it forms an alpha polymorph, in which randomly distributed silver ions (often described as being “partly melted”) can move through the solid iodide lattice when provoked by an electric field.
SSE research and technology has come far since these early days and today there are broadly two types: Solid Inorganic Electrolytes (SIEs) and Solid Polymeric Electrolytes (SPEs). Both types of SSE, of course, pass the entrance exam for use in batteries: facilitating ion transport through their bulk, whilst being electronically insulating. However, their specific compositions endow them with a range of strengths and weaknesses which must be leveraged and mitigated, respectively. These characteristics are briefly summarised below.
SSE Type | SIE | SPE |
Composition and Mechanism | Crystalline or glassy inorganic solids which allow for ionic diffusion through their lattice, often down 1D channels or 2D planes. | SPEs comprise a solvent free salt solution in a polymer host material that conducts ions along the polymer chains, often through interaction with polar groups. |
Positives | Good ionic conductivities, high moduli, good thermal stability and wide electrochemical stability windows. | Easy synthesis and processing, low mass density, low cost, good chemical stability. Allow metal anode use and therefore energy dense. |
Negatives | Hard to manufacture. Poor interfacial charge transport with electrodes, dendrite growth along grain boundaries, high cost and poor environmental stability. | Low dielectric constant and low ionic conductivity. |
Examples | beta – Al2O3; perovskite; anti-perovskite; NASICON (NaZr2(PO4)3); sulfide (Li10GeP2S12); hydride (Li2(BH4)(NH2); garnet (Li7La3Zr2O12) | PEO, PVDF-HFP, ABA block polymers; crosslinked polymers; single ion polymers. |
Source: Zhao, Q., Stalin, S., Zhao, CZ. et al. Designing solid-state electrolytes for safe, energy-dense batteries. Nat Rev Mater 5, 229–252 (2020). https://doi.org/10.1038/s41578-019-0165-5.
The distinctive properties of SIEs and SPEs makes the development of composites an attractive option; the hope being to balance the strengths and weaknesses of the individual chemistries.
Such combinations can even produce synergistic effects. For example, the addition of inorganic particles into the bulk of an SPE such as PEO can increase mechanical strength, improve thermal stability and prevent crystallisation (allowing for improved ion transport). It is also suggested that well connected inorganic regions can themselves aid ionic conductivity.
The use of SSEs can also enhance the raw electrochemical performance of a battery. Whilst not trivial to do, the solid nature of the electrolyte can permit the use of metallic lithium as an anode, meaning higher energy densities can be achieved. This is made possible by the solid nature of SSEs preventing the growth of metallic lithium microstructures known as dendrites that form on the anode when ions cannot be absorbed into the bulk of the electrode fast enough.
Further, it is not just the ionic conductivity of an electrolyte that matters, but also what is doing the conducting. The transport number of an ion in an electrolyte describes how much of the current it carries as the battery is charged and discharged. When an electric field is placed across a typical electrolyte the Li+ ions go one way and the PF6- ions the other, each carrying a fraction of the charge transferred (e.g. t+ = 0.3, t- = 0.7). The sharing of the charge transport between ions like this can lead to polarisation at the electrode interfaces and limit performance. SIEs, in contrast, are single ion transport media and so Li+ (although research is ongoing into the use of many other ions) would carry all of the charge.
In broad terms, SSEs promise improved stability, performance and safety (notably reduced flammability as no solvents are needed). However, there are still many hurdles to overcome. For example, SSEs can exhibit poor interfacial kinetics due to inferior electrolyte/electrode contact and unwanted interfacial reactions, and there are many challenges with processing at scale.
The surge in interest directed towards SSEs can be largely attributed to the demand for high performance electric vehicles. Electric vehicles require batteries that are energy dense, powerful and have good cyclability (for greater range, faster charging and longer life-span, respectively).
Theoretically, the use of SSEs could contribute to the attainment of all of these features. Because of this, interest in the development of SSEs spans from fundamental research right through to commercial application.
On the latter, a notable example is that of VW investing $300M into QuantumScape, a secretive silicon-valley start-up developing lithium-ceramic solid-state batteries showing impressive performance in recent independent tests and promising dramatic improvements over current Li-ion technology. Ford and BMW have made similar investments in Solid Power Inc. and Toyota were the single biggest proprietor of solid-state battery patents from 2014-2018.
We have a lot to thank the current Li-ion battery for; it has become so widely and deeply embedded into our every-day lives that it is hard (certainly for those of my generation) to imagine a world without it.
However, the world is imposing constraints and painting new targets for batteries that necessitate step changes in all aspects of its design. There is a buzz of activity in all areas of research and development surrounding batteries; the use of solid-state electrolytes is just one example of a technology that can help make batteries the tool we need them to be for a sustainable and fair future.
Fynn is a trainee patent attorney in the chemistry team. Fynn has a Master's (MChem) degree from the University of Oxford. During his undergraduate degree, he completed research projects modelling battery cathode materials and ion transport as well as ab initio modelling of quantum tunnelling processes. His thesis centred around simulating the ultrafast dynamics of photo-excited, conjugated polymers and developing novel ways of extracting information through experiment.
Email: Fynn.McLennan@mewburn.com
Our IP specialists work at all stage of the IP life cycle and provide strategic advice about patent, trade mark and registered designs, as well as any IP-related disputes and legal and commercial requirements.
Our peopleWe have an easily-accessible office in central London, as well as a number of regional offices throughout the UK and an office in Munich, Germany. We’d love to hear from you, so please get in touch.
Get in touch